PASADENA, Calif.—Researchers from the California Institute of Technology (Caltech) have been able to view in detail, and for the first time, the previously mysterious process by which long chains of a protein called ubiquitin are added by enzymes called ubiquitin ligases to proteins that control the cell cycle. Ubiquitin chains tag target proteins for destruction by protein-degrading complexes in the cell.
"We found that ubiquitin ligases build ubiquitin chains very rapidly by transferring ubiquitins one at a time," says Raymond Deshaies, professor of biology at Caltech and Howard Hughes Medical Institute investigator.
Their findings, and the innovative process by which they were obtained, are described in this week's issue of the journal Nature.
Ubiquitin is one of nature's most unusual proteins. Unlike most of its protein brethren, ubiquitin has to be physically attached to other proteins to do its job.
“As its name implies, ubiquitin is found in essentially every kind of eukaryotic cell," says Caltech graduate student Nathan Pierce, the Nature paper's lead author.
In their Nature paper, the Caltech team looked at the process of ubiquitylation, the method by which ubiquitin and ubiquitin chains are added to target proteins. The target proteins used in the study, cyclin E and β-Catenin, are both involved in controlling the cell cycle.
It was already known, Pierce explains, that the addition of a chain of four or more ubiquitins to a target protein marks that protein for annihilation.
The destruction of cyclin E is critical for the accurate replication of DNA, while the degradation of β-Catenin keeps cells from dividing during development at the wrong time. If β-Catenin is not degraded, cells proliferate excessively and become predisposed to tumorigenesis. Meanwhile, cells that don't degrade cyclin E accumulate DNA damage and mutations, which can help fuel the unchecked growth of a tumor.
It was also already known that ubiquitin chains are added to the protein using three different enzymes, dubbed E1, E2, and E3. Simply put, E1 activates ubiquitin for transfer, then passes it over to E2. E3 then gets into the act. A form of E3 called a RING ligase (RING stands for "really interesting new gene") plays a key role in the tagging of cyclin E and β-Catenin; according to Pierce, the RING ligase "simultaneously binds to E2 and the target protein (like cyclin E), and then causes E2 to transfer the ubiquitin to the target protein."
Despite all of this knowledge, one question has remained: is the chain transferred to the protein in an already assembled form, or are the ubiquitins moved over one at a time?
"The process is so complicated and so fast," Pierce notes, "that we weren't able to see how the chain is actually built."
To address that issue, Pierce created a sort of biological stop-motion animation that allowed the Caltech team to watch every step in the transfer of ubiquitin from E2 onto the cyclin E protein substrate.
"We devised methods to take snapshots of ubiquitin ligase reactions at a rate of up to 100 'pictures' every second," says Deshaies. "This enables us to see things that would normally evade detection. "
Previous studies had looked at the reaction on the scale of seconds or minutes, Pierce adds. But through an innovative use of a laboratory tool called a quench-flow machine—a machine that allows for extreme precision in the stopping, or "quenching," of a reaction—the team was able to look at what was going on over intervals of just 10 milliseconds in both yeast and human proteins.
"Prior methods did not have sufficient time resolution to see what was going on," says Deshaies. "It's as if you gave an ice-cream cone to a kid and took pictures every minute. You would see the ice cream disappear from the first photo to the next, but since the pictures are too far apart in time, you would have no idea whether the child ate the ice cream one bite at a time, or swallowed the entire scoop in one gulp."
The new method revealed the biological equivalent of small, single bites of ice cream. "Using our approach," Deshaies says, "we could see that our ubiquitin ligase builds ubiquitin chains one ubiquitin at a time."
"Once we knew what the steps were, we calculated the rates at which they occur," adds Pierce. "And from those rates, we were able to really describe the biology of how this system works."
The quest doesn't stop there, of course. "One thing we have to understand now is, how do ubiquitin ligases achieve the speeds that they do?" asks Deshaies. "What special mechanisms do they have to enable them to build chains rapidly? And the flip side of the coin: What sets the speed limit? Why can't our ubiquitin ligase work even faster?"
A recent paper published in the journal Cell by Gary Kleiger, a postdoctoral scholar in the Deshaies lab, answered some of these speed-related questions. By measuring the rates at which E2 and E3 interacted with one another, Kleiger was able to demonstrate their unusually fast association—faster than predicted for normal proteins. E2 and E3 use oppositely-charged surfaces to attract each other, thereby speeding up the formation of a functional complex of the two proteins. This helps explain how the rapid sequential additions of ubiquitin described in the Nature paper are possible.
Gaining these kinds of insights into the ubiquitin system is important, Deshaies says, because ubiquitin ligases play a critical role in a number of human diseases, including cancer, due to their role in the regulation of the cell cycle.
"Once we understand these aspects of how ubiquitin ligases work, and what limits their speed, we will be in an excellent position to think about how we might develop drugs that attack the ligase's Achilles' heel, to make its slowest step even slower," he says. "If we can slow down ubiquitin ligases enough, they may become too slow to get their job done—to build chains—in the time available to them to do so. Being able to develop drugs to block their function would open up a new frontier in medicine."
"We were able to invent HIV therapeutics because we understand how reverse transcriptase works," adds Pierce. "The same applies here. We need to understand how these enzymes work if we're ever going to be able to target them with therapeutics."
In addition to Pierce and Deshaies, other researchers involved in the study included Kleiger and Shu-ou Shan, assistant professor of chemistry at Caltech.
The work described in the Nature paper, "Detection of Sequential Polyubiquitylation on a Millisecond Time-Scale," was funded by a Gordon Ross Fellowship, National Institutes of Health training and research grants, and the Howard Hughes Medical Institute.
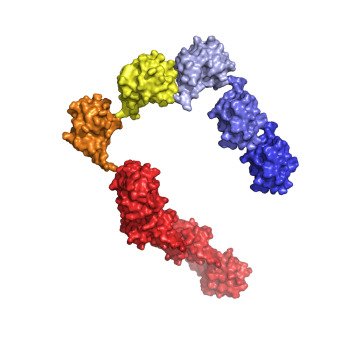
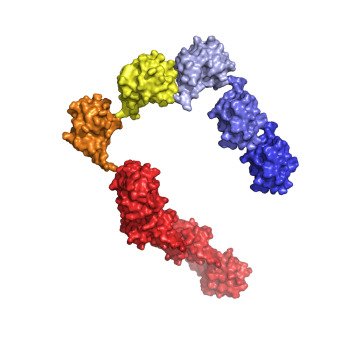
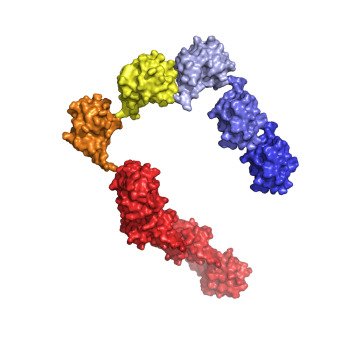
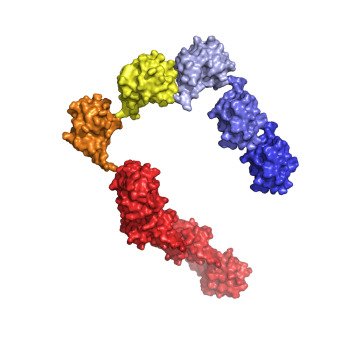